This is an excerpt from the book series Philosophy for Heroes: Act.
To understand human nature, we can compare ourselves with our closest primate cousins, the chimpanzees. How do we differ, what makes us special, and what has taken us on two different paths?
Some 66 million years ago, a 10km- to 15km-wide asteroid hit what we today know as the Gulf of Mexico and in its aftermath killed all animals over 55 pounds body weight (the Cretaceous-Paleogene extinction event). This opened an opportunity for the smaller mammals to slowly return from their nocturnal life. Prior to this fateful event, mammals had adapted by hiding during the day. At night, they relied on their sense of smell to evade predators and to locate prey. This led them to evolve abilities that turned out to be useful when they returned to the daylight. One of those abilities was suppressing immediate urges. With the help of the prefrontal cortex, the mammal was able to save valuable time and energy. It could ignore scents of predators that were long gone, and pursue prey that was still nearby.
Prefrontal cortex The prefrontal cortex is part of the frontal lobe and can be understood as running a simulation of the world. It monitors social relationships, keeps track of objects when they are no longer visible (object permanence), and helps with the pursuit of long-term goals. It has only indirect connections to brain parts dealing with actions or sense perception.
When mammals later adapted to the daylight, the same principle was applied to visual data. For example, just seeing a lion does not mean that the lion is dangerous; it might be sleeping. To make that judgement call, the separate evaluation and signal by the prefrontal cortex was needed as a counterbalance, thinking long-term, balancing risk and reward.
The prefrontal cortex is only indirectly connected to any sensory organs or muscles, hence it is believed to process thoughts that do not need any external input to be activated and do not necessarily result in a concrete action to be executed. Put differently, the prefrontal cortex reads and controls the rest of the brain instead of directly accessing sense organs or muscles.
To understand the prefrontal cortex’ role, we need to remember that many parts of the brain are in competition with each other. In that regard, the prefrontal cortex can be understood as a counterbalance to the rest of the brain. Applications include long-term goals, social rules, hidden threats, imagination, or memories. For example, a strong visual input like a tiger standing in front of us would need an equally strong competing signal to prevent a simple fight, flight, fawn, or freeze reaction.
The Primary Motor Cortex
The first true primate ancestor was a small nocturnal monkey-like, forest-dwelling animal (Plesiadapis) that lived in trees and ate fruit (around 55 million years ago). This primate-like mammal needed strength and balance to jump from tree to tree, and a brain that could calculate movement in three-dimensional space, as opposed to just navigating on the ground. Also, its eyesight had to improve to adapt to the light of day and to recognize ripe fruit. A testament to this evolutionary history is that we now lack the ability to produce our own vitamin C and need to rely on a steady diet of fruit and vegetables.
About 30 million years ago, the evolutionary branch split into Old World monkeys and New World monkeys. The most widely held theory about this split is that the ancestors of the New World monkeys used a temporary land bridge or series of islands between Afro-Eurasia and South America. The main difference between the two types of monkeys is that New World monkeys kept their tail. With their primary motor cortex being focused on their tail muscles, they use it like a fifth limb. In contrast, the primary motor cortex of apes (Old World monkeys) is specialized for hand use which helps with foraging for fruit. To observe this in humans, just stand in a modern supermarket and watch people carefully checking out the ripeness of avocados.
Primary motor cortex The primary motor cortex is part of the frontal lobe and is directly adjacent to the primary somatosensory cortex of the parietal cortex. This way, it can directly process data from our sense of touch to better control movements. The primary motor cortex connects to the brainstem and spinal cord (via the upper motor neurons) which in turn connect to the muscles (via the lower motor neurons).
The feature that makes the primary motor cortex in primates special compared to that of other mammals is that it can bypass the spine’s interneurons (the spine’s relay station) and send signals directly to the motoneurons of the spine [Rathelot and Strick, 2009]. With a significantly higher number of neurons controlling the movement, this allows fine-grained control of the actual signals sent to the muscles. This part of the brain also grew overproportionally during the evolution of primates [Rowe et al., 2011, cf.].
Why exactly do more neurons lead to better control?
Our primary motor cortex contains about 5 billion neurons controlling some 600 muscles. Those 600 muscles consist of more than 50 billion muscle cells. But muscles can only contract, so, theoretically, to contract all 600 muscles individually, no more than 600 motor neurons would be needed. Signals arriving in the primary motor cortex already contain the specific motor program, so all that is left for the primary motor cortex to do is to translate those into signals associated to individual muscles.
Let us imagine two extreme architectures:
- If any nerve detects a signal, all muscle cells contract. This is the case with the nerve net in the previously discussed Hydra. As fewer motor neurons need to be activated, this option is extremely energy-efficient and quick: each movement could be done with maximum strength in the blink of an eye. Theoretically, you would need only a single nerve cell to control all muscles in your body. On the other hand, the only possible action you could take is to contract all muscles at the same time. Imagine you could fully exert your biceps with the same mental effort it takes to move your little finger. You would leave the gym with sore muscles but mentally, you would still feel refreshed. The downside is that you would not have any precision if all you could do is exert full force or exert no force at all.
- Each muscle cell is connected to an individual nerve cell coordinating whether or not it contracts. A movement can involve any number of muscle cells in any combination and sequence, allowing very fine-grained control of the position of the limbs as well as the force exerted with each movement. This option is very energy intense, though. Not only would your body have to provide energy for your muscles to work, your nerve cells would take a similar amount of energy to operate the muscles. Our brain uses around 10 billion neuron cells to control 50 billion muscle cells. To reach a level of control where one neuron cell controls one muscle cell, our brain would have to be around 50% larger than its current size (our brain has around 86 billion neurons and would need around 126 billion neurons).
Our own primary motor cortex resembles the latter architecture more than the former. Compared to our more powerful cousins, the chimpanzees, our primary motor cortex is about 20% to 70% larger [Donahue et al., 2018]. In chimpanzees, the relatively small number of motor neurons can be activated very quickly, allowing for greater strength. On the other hand, the higher number of motor neurons in humans allows for more precise movements and significantly less energy expenditure by the muscles. This gives us better control over how much force we want to exert—a crucial ability when it comes to using (or producing) more advanced tools like bows or spears [Walker, 2009].
The Rise of the Hominids
Further splits occurred about 15 million years ago (gibbons), 13 million years ago (orangutans), and 10 million years ago (gorillas), with the final split from chimpanzees and humans between four million and 13 million years ago. From there, we can trace back our human ancestors, with their most distinctive feature being a progressively increasing brain volume:
- Australopithecus (3.6 million years ago, 485 cm3cm3)
- Homo habilis (2.1 million to 1.5 million years ago, 650 cm3cm3)
- Homo erectus (2 million to 0.14 million years ago, 1100 cm3cm3)
- Homo heidelbergensis (0.7 million to 0.2 million years ago, 1230 cm3cm3)
- Homo neanderthalensis (250 thousand to 40 thousand years ago, 1500–1740 cm3cm3)
- Homo sapiens (today, 1425 cm3cm3)
With Neanderthals having had larger brains than humans, were they more intelligent than humans?
To answer this question, it is important to note that besides humans and their ancestors, other animals also have large brains. For example, bottlenose dolphins have a similar brain size as humans have. They have been observed helping out other dolphins as well as those of other species like humans (e.g., drowning divers), which points to them sharing our ability to understand what other beings are possibly thinking or experiencing [cf. White, 2007, p. 41]. But to compare brain sizes between humans and other animals, we need to include their body size into our calculation. Larger bodies produce a larger quantity of signals that need to be processed and have more muscle cells that need to be activated. For example, while whales have a brain size more than five times that of humans, they are not necessarily quick (or creative) thinkers. They need that brain size to control the muscles in and the sensors of their huge body (around 50 tons). Thus, to get a sense about an animal’s intelligence, we need to look at the total brain size divided by the body mass, and set it in relation to the average (for the species) brain size and body mass. This so-called encephalization quotient (EQ) provides such a mapping, making it possible to compare different animals’ potential intelligence (Roth and Dicke [2005]).
Encephalization quotient The encephalization quotient (EQ) is a measure of relative brain size and is often used to convey how small or large a species’ brain is compared to that of other species of similar body size.
When accounting for their larger body mass, the encephalization quotient of Neanderthals is similar to that of modern humans. And it has been shown that Neanderthals created and used tools like spears, possibly had language, and developed their own cultures. So, how do we differ from Neanderthals?
We find a clue by looking at gorillas. With an encephalization quotient of 1.5, they are at the lower end of the EQ spectrum—while clearly highly intelligent given that they can learn sign language and make and use simple tools. This points to the EQ not telling the entire story. Hence, scientists have divided the brain mass further into parts necessary for the maintenance and control of the body and senses, and those associated with improved cognitive capacities [Roth and Dicke, 2012]. Subsequent studies on Neanderthal brains [Pearce et al., 2013] have shown that they must have had significantly larger eyes (possibly to allow better sight for hunting where there was not much light) than those of humans. Also, the brain part responsible for image processing must have been larger, which left less brain mass for social relationship processing. The currently accepted theory is that humans used their social intelligence as an advantage over Neanderthals, ultimately replacing them—even though the latter possessed better sight and body control. Research seems to point to multiple migrations from Africa to Europe [Kuhlwilm et al., 2016] and back from Europe to Africa [Chen et al., 2020] with interbreeding over a prolonged timespan.
Further research needs to be done in regard to arthropods (insects, spiders, etc.) and cephalopods (especially cuttlefish, squid, and octopodes). For example, jumping spiders are able to plan ahead and to apply hunting strategies, and even care for and nurse their young like mammals do [Chen et al., 2018]. This is reflected in their brain-to-body ratio: a jumping spider’s brain requires so much space that it is distributed throughout its tiny body. Another example would be octopodes; they have two-thirds of their nervous systems in their arms, resulting in a brain-to-body quotient comparable to that of humans. Octopodes can use tools, solve puzzles, recognize people, and plan ahead.
In the case of some animals, to counter the size limitations of the brain, the brain uses cortical folding to increase surface area and processing speed [Striedter et al., 2015]. Imagine that very early during embryonic development, the neurons form a flat plane and then fold while the surface area is growing. This is comparable to creating towels by adding loops of thread (the folds) to a piece of cloth. They increase the surface area of the cloth without increasing the size of the cloth (the plane). Trees solve the problem of capturing sunlight in a way that is similar to the brain creating folds: to maximize the exposure of leaves to the sun, the tree creates branches which subsequently create branches and so on. This way, the distance from each leaf to the trunk is limited, while maximizing the amount of sunlight the tree can capture (see Figure 5.9). Objects like these are called fractals (see Philosophy for Heroes: Continuum ).
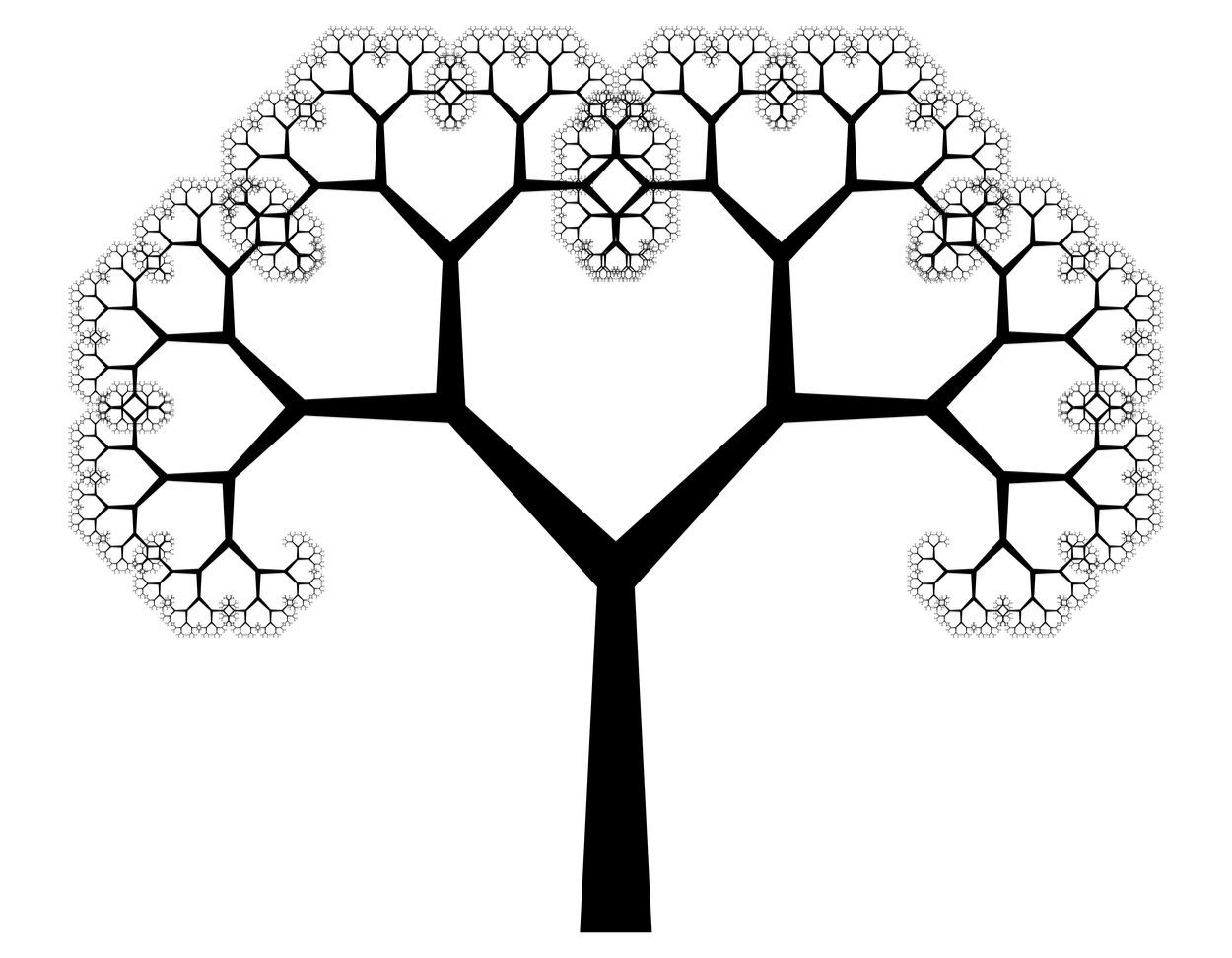
The Rise of the Humans
How could larger brains have helped early humans to survive?
After all, we apply most of our intelligence to utilize complex language and tools. But those things were not available to early humans. And compared to other mammals, even compared to apes, humans are neither strong nor fast, we do not have body armor, we do not have claws or poison, we do not have wings or a strong sense of smell, and we cannot see well at night. At the same time, larger brains meant a higher energy need. In that regard, the question of how human intelligence evolved looks like another chicken-and-egg problem similar to the question of the origin of life (see Philosophy for Heroes: Continuum ). We need to find an evolutionary path of incremental genetic changes, benefiting our ancestors at each step.
We have become a complex product of nature because our ancestors had to adapt and re-adapt again and again to ever-changing environments. Compare that to lifeforms that have not changed significantly for hundreds of millions of years: they have found a niche in which there was no evolutionary pressure to adapt to new challenges.
The important lesson is that evolution does not work in a directed way where all lifeforms become smarter over time. Brains are simply an adaptation to very specific problems. Each part of our brain addresses a particular need to process information in order to give us an edge over our predators and prey.
Hence, one approach to the question of how humans have diverged from other primates is to think about the influence of the environment on our ancestors’ evolution. The split from the chimpanzees four million years ago probably happened between those of our ancestors staying in the forest and those going out into the savannah. As trees provided protection and sources of food, it is possible that the savannah was not their first choice. Perhaps a change in geology or climate caused the forest to recede. Later, population growth might have driven our ancestors out into the savannah; this is the savannah hypothesis.
Savannah hypothesis The savannah hypothesis states that early humans evolved on the savannah and that many of the modern human’s traits are a result of this adaption.
While the precise sequence of events is still debated—more recent evidence points to a much less abrupt transition from forest to the savannah [Domnguez-Rodrigo, 2014]—the following traits were conducive for early humans to survive in the savannah:
Fire and cooking. Fire allowed early humans to be more active at night. It provided warmth and helped to fend off predators. With the ability to control fire, early humans were able to cook food. This reduced the time needed for digestion compared to eating raw foods, freeing up energy for the brain. While in other apes, the colon represents about 50% of gut volume, in humans, it is less than 20% [Furness et al., 2015].
Bipedalism. Bipedalism probably developed before our human ancestors moved into the savannah. After all, apes are able to walk on two feet; they typically choose not to do so. Their muscle configuration makes walking less energy-efficient when compared to humans—just like we could walk on our hands, but it takes far too much energy [Sockol et al., 2007].
Endurance. Better muscle control due to the larger motor cortex allowed our ancestors to travel longer distances—a very advantageous trait for living on the savannah.
Cardiovascular system. We see additional optimization toward endurance in our heart which has adapted for moderate-intensity activity like walking, hunting, or farming. (By contrast, chimpanzees’ hearts are optimized for short bursts of intense activity such as climbing and fighting, see Shave et al. [2019]). This enabled our human ancestors to cover greater distances in the savannah, spreading their genes with tribes farther away. And while we cannot run faster than a cheetah in a sprint, we can outrun it in any hunt longer than a mile. Cheetahs are all about sneaking close to their prey and then sprinting toward it in one short burst.
Sight. In the forest, chimpanzees’ main visual focus is spotting details. The global image of the forest as a whole is less important than identifying, for example, a snake, or fruits, or another chimpanzee hiding among the leaves. By contrast, the focus of humans adapted to a life in the savannah is a more comprehensive, global image. To coordinate a hunt, you need to keep your entire environment in mind and create a plan for how to approach prey from different directions. Studies have shown that humans are much better than other primates at integrating local visual information into a global whole [Denion et al., 2014]. Also, being on watch for predators or coordinating a hunt requires humans to constantly scan all directions. The anatomy of our eye sockets gives us a wider field of view compared to that of chimpanzees [Imura and Tomonaga, 2013]. This is also more economical as we have to move only our eyes rather than our entire head.
Ranged weapons. It takes precision and perseverance rather than explosive strength when looking at an animal from a distance, feeling the wind, taking a wooden spear, calculating which of the dozens of muscles to stretch and release and in what sequence so that the stick hits its target, and then tracking the wounded animal over hours. In order to throw accurately, the brain has to calculate a sequence of nerve pulses to coordinate which of the hundreds of muscles should contract and in what order. To maximize the impact of a throw, the muscles have to work in harmony, just as performers in an orchestra play various instruments and create a harmonious sound. The comparison between throwing a spear and playing music is fitting as the same regions of the brain are used to produce both. While ranged attacks can be found in nature (tongues of chameleons, other primates throwing sticks and stones, archerfishes spitting water at bugs, pistol shrimps “shooting” air, jumping spiders jumping at their prey), humans are the only animal in nature to accurately throw things at long distances.
Language. Building a sentence requires advance planning and coordination. We need to use words in a specific order to carefully construct the sentence rather than trying to exert ourselves in one loud call. This uses the same mental machinery that is required to accurately throw. The cognitive trade-off hypothesis explains this difference between chimpanzees and humans by stating that our human ancestors exchanged (some of) their short-term memory for other abilities like abstract language and planning. Evidence for this is in (compared to chimpanzees) humans’ significantly larger angular gyrus, which helps with tool use, reading, and language [Fjell et al., 2013]. It is also home to our working memory (at least the phonological memory), which is superior in humans compared to chimpanzees [Read, 2008].
Reaction time and memory. While humans indeed require short-term memory for conversation, remembering telephone numbers, and reading, only a minimum amount is needed. Not only is a conversation—compared to the snap decision a chimpanzee has to make—stretched out over an extended period of time, but there is also always the possibility of asking questions.
Our ancestors in the forest faced a situation very different from those who went out in the savannah. In the forest, with trees blocking their line of sight, they had less time to react to sudden encounters with predators (or rivals). At the same time, they could use the trees to flee from their predators. This adaptation to quickly evaluate and react to a situation seems to be reflected both in today’s forest-dwelling chimpanzees’ strength as well as in their (compared to humans) superior short-term memory capacity. Experiments have shown that some chimpanzees need only 0.21 seconds (compared to humans needing at least 0.65 seconds) to remember the position and sequence of nine numbers on a computer screen [Inoue and Matsuzawa, 2007]. This is similar to what a chimpanzee might encounter in the wild: imagine nine rival chimpanzees showing up. For the one chimpanzee defending itself, each second spent determining whether to flee to the trees or to prepare to fight might determine whether or not the chimpanzee loses territory, is injured, or even killed.
Games. Language also allows more educational play. While other animals (for example dogs or their puppies) have ways of signalling they want to play-fight, humans can develop much more complex games (e.g., hopscotch, fencing, martial arts, soccer, chess, etc.) to allow them to train their mental and physical abilities [Kerney et al., 2017]. We can even use play productively: for example, we train people to operate in space while they are still safely on Earth.
Complex tool use. The mental machinery required to process language also allowed us to create complex tools. Sentences consist of subjects, objects, verbs, adjectives, and adverbs that connect with or modify each other. Similarly, tools consist of different objects that need to be connected. If you can imagine sentences that describe how entities interact with each other, you can imagine tools consisting of entities interacting with each other. Indeed, we can rely solely on language to describe the production of, for example, a hand-axe or spear. While tool use is not something unique to humans, we have seen only a few examples in the animal world. For example, some monkeys have learned to dry certain nuts, and then later use specific stones to crack them open—a skill that can take years to learn properly [Luncz et al., 2017]. But monkeys are probably not able to create something as complex as a bow and arrow.
Weapon evolution. Over time, our ancestors developed better and better weapons. For example, they added a sharp stone for additional weight, impact damage, and flight properties, and thus, invented the stone-tipped spear. Accurate ranged weaponry offered significant advantages to our ancestors. Not only did they become better at hunting, but also the prey’s own defensive weaponry including hooves, claws, and fangs became useless against humans who were no longer within reach. Archaeological evidence puts spear use by our ancestors at as early as 500,000 BC [Wilkins and Chazan, 2012]. This led to prey animals becoming more cautious. Individual animals that were more anxious had an evolutionary advantage over more courageous or curious animals. Thus, with each generation, they tried to stay farther away from anything that resembled a human. This put evolutionary pressure on humans to produce better and better tools, throw farther, and invent increasingly intricate hunting techniques and strategies. This created a predator-prey dependency. Like the evolution of the eye (see Philosophy for Heroes: Continuum ), improvements in our ability to make and throw projectiles brought us an advantage at every step of the way.
Self-domestication. Chimpanzees are much more aggressive than humans, but they are also more hesitant to go into a fight, given that it comes with a significant risk of injury. Humans, on the other hand, evolved the ability to attack from a distance, attack together with others, and even to plan an attack in advance. This put anyone at risk, no matter his or her status. Anyone could be challenged as long as there was support from other members of the tribe. This led to significant changes within tribes of humans: the most aggressive or anti-social members of the tribe could be singled out more easily. Just like we domesticated wolves by selecting the least aggressive pups from a litter, humans self-domesticated by removing the most aggressive members of their tribe. While (for the most part) peaceful within the tribe, humans became efficient hunters for everything outside of their tribe. We still have to grapple with this dual nature of humanity—sharing a strong sense of community, while also expressing an “us versus them” mentality. On the one hand, we can easily make peace with the people around us; on the other hand, we can rationalize (by mentally degrading them) killing animals and killing humans from other tribes. While we might look down at chimpanzees for their in-the-moment aggression and impulsivity and see ourselves as the pinnacle of evolution, we also have to remember how easily humans can suppress their empathy once they have judged someone as “sub-human.”
Interestingly, studies have shown that in mammals, certain genes regulate both aggressivity and aspects of anatomical features of the face. This genetic connection explains why we have shorter faces and smaller teeth compared to our primate cousins. Similarly, many domesticated dogs look less threatening than wolves. If our ancestors did not specifically breed wolves, it is conceivable that the least aggressive (and thus also least aggressive-looking) wolves were able to approach a human camp without being attacked by humans. A connection between behavior and facial structures can also be seen in humans. People who are missing a copy of the BAZ1B gene have the Williams-Beuren syndrome and are more talkative, outgoing, and less aggressive. They also have rounder faces with shorter noses, full cheeks, and wide mouths with full lips. Vice versa, people with additional copies of the BAZ1B gene (the 7q11.23 duplication syndrome) tend to be aggressive, have difficulties socializing, and their facial features are also affected [Zanella et al., 2019].
Rules and laws. Over time, human civilization has replaced the lethal way we deal with aggressive members of our tribes with methods of coping with our emotions: culture, customs, rules, and ultimately, laws. Those of our ancestors who were able to reflect upon their own status and position within society, and how it might feel to be another member of society, ultimately prevailed. As the anthropologist Richard Wrangham put it, “Those who followed the rules were favored by evolution” [Wrangham and Grolle, 2019, cf.].
Compared to our primate cousins, humans are actually much less eager to change the pattern of how they carry out a task [Watzek et al., 2019]. In a study, the participants—humans, rhesus macaques, and capuchin monkeys—had to select three icons in sequence to score a point (or get a banana) in a set of 96 trials. The third symbol showed up once the first two were selected in the correct order. In the second set of the trials, the third symbol showed up immediately; participants could either select all three symbols in order or select only the third symbol to get the reward directly. Most monkeys switched to the more efficient strategy of selecting the third symbol without delay, while humans tended to stay with their previous strategy. Preference for the familiar over a new approach was also confirmed in a second study comparing humans with chimpanzees [Pope et al., 2020].
Protected childhood. Compared to babies of other mammals, human babies are totally dependent on their parents. This becomes obvious when comparing human babies to chimpanzee babies: without their mother, human babies are helpless, while chimpanzee babies are at least able to follow or hold onto their mother from early on. Intelligence-wise, human children catch up with chimpanzee children only at the age of one or two years. This is because the brain of a human baby continues to proliferate after birth; by contrast, in terms of growth, chimpanzee brains level off very soon after birth. We should not look at prolonged childhood being an evolutionary mishap or obstacle, though. Instead, it is more a testament to the success of human parents to provide the necessary protection and nutrition during the earliest periods of their children’s lives. It is an expression of the long-term investment into the brain development of the child.
Giving a baby the safe space to develop his or her brain without the need to survive independently gives humans an evolutionary edge. It is easier for the brain to put down new neural pathways in a part of the brain that is not being used at the time, just like it is easier for a construction company to replace rail tracks when no trains are using them. We have to keep in mind that we cannot just shut down our brain for a few days for architectural changes. While some cleanup processes happen during our sleep, we still have to be ready to respond within seconds when being awakened by, for example, the sound of a possibly dangerous animal or another human. The requirement to care for our children seems to be another evolutionary factor driving our longevity. As grandparents, we can spend resources on caring for our grandchildren or other relatives. This is not exclusive to humans; it has been shown that among killer whales, post-menopausal whales provided significant survival benefits for their grand-offspring [Nattrass et al., 2019].